Decoding cellular diversity in the nervous system
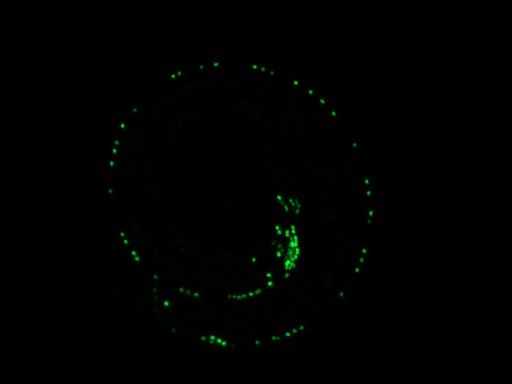
The C. elegans roundworm has just 302 neurons (highlighted in green) which allows scientists to study how they distinguish their identity. (Photo by Manasa Prahlad, Graduate Student, Genetics, Genomics and Systems Biology)
Our bodies are made up of billions of cells, all working together to keep us alive and functioning. To do this, they rely on unique gene expression patterns, molecular signatures that distinguish each of them in form and in function. If these signatures are disrupted it can cause devastating diseases like cancer.
Maintaining these signatures can be more challenging for some cell types than for others, however, depending on the cell’s lifetime. For example, while cells in your intestinal lining turn over every five days, and your liver cells every year, the neurons of the central nervous system, which you use every second to think and to move, last your entire life! This means that, unlike rapidly regenerated cells, neurons need to have tightly regulated mechanisms in place to maintain their specific cellular identities.
Motor neuron disease causes loss of neuron identity and function
I’m a graduate student in the lab of Paschalis Kratsios, PhD, Associate Professor of Neurobiology at the University of Chicago. Our research is particularly interested in studying how neurons develop their distinct molecular signatures and cell identities. This is because the development of neurodegenerative diseases, such as amyotrophic lateral sclerosis (ALS), Alzheimer’s disease, and Parkinson’s disease, involves the disruption of important genes that neurons need to function. This consequently causes the neurons to be lost altogether. Neurodegenerative diseases like these are devastating because they directly affect the central nervous system (CNS), i.e., the brain and the spinal cord, forever impairing cognition and locomotion.
To form a functioning nervous system, it’s essential that you have neurons of different types, performing different roles. In our lab, we want to understand how different neuron types express different sets of genes. We study this question in the nematode C. elegans, a millimeter long transparent worm (I promise, they’re cuter than they sound). In contrast to the hundred million neurons in the human nervous system, C. elegans has only 302 neurons. This allows us to examine the mechanisms of neuron identity in a simplified context.
Since our lab is part of the UChicago Center for Motor Neuron Disease, we also study models of ALS by focusing on the 75 motor neurons located in the worm’s ventral nerve cord – similar to our spinal cord. These neurons, which the worm uses to move, are arranged almost single file in a line extending from the worm’s head to its tail. And even this subset of neurons isn’t all the same. But how do we know this?
What is cell identity?
I wrote earlier that a cell’s molecular signature, or pattern of gene expression, indicates its identity. And with today’s rapidly developing techniques for studying individual cells’ signatures in detail, it has become standard to consider a cell’s molecular signature and its identity as synonymous. Sequencing whole cell RNA has only been possible for around a decade, however, and is still a very expensive process. For the many decades before single-cell sequencing technology, scientists had to be creative to first describe unique cell types, and then piece together the genetic elements, the molecular signatures, that give them their unique identities.
C. elegans has been popular among nervous system researchers since it was established as a model organism in the 1970s. At the time, they used high-contrast light microscopy and electrophysiological experiments to characterize motor neurons based on their neurotransmitters, their connecting partners, and the shape of their axons. With just these criteria, they were able to define seven distinct motor neuron types – a classification still used today. But to determine the invisible gene regulatory decisions that lead to visible physical distinctions between motor neuron types, researchers learned to knock out various genes at random and monitor the visible effects that those mutations had on motor neurons. This was the beginning of the story, in which the most essential genes for neuronal development were identified.
In our lab today we do much the same thing, using microscopes and genetic mutants to probe the intricacies of neuron development. However, with the advent of bright fluorescent reporters (like GFP, first introduced in 2008) and precise DNA-editing technologies (like CRISPR, 2012), our job is much easier. Now, we don’t just look for major defects. We can directly monitor gene expression levels from microscopy, and thus figure out how different genes interact. In other words, we can make those previously invisible genetic relationships visible, refining our understanding of the complex networks that create specific molecular signatures.
Combining old and new methods to explore new questions
Recently, our lab took the next step, the painstaking process of collecting tens of thousands of C. elegans motor neurons, extracting and sequencing their RNA. Now, what can we do with this rich dataset of millions of RNA sequences?
As powerful as our single-cell RNA-sequencing (RNA-seq) dataset is, it would have been extremely difficult to interpret without our prior knowledge of some of the molecular differences between cell identities. But by combining our experimental data with this massive sequencing dataset, we mined this new resource to determine novel genetic markers, or new pieces of molecular signatures, for each cell type. This alone is a huge advance towards understanding how different neuron types are affected in neurodegenerative disease.
By uncovering these new molecular markers, we can also identify more subtle trends in neuronal diversity. For example, while our RNA-seq data showed that the original seven motor neuron types are indeed molecularly distinct from one another, we found that each of these identities could be subdivided into even more molecularly distinct subtypes. More importantly, these subtypes correspond to a neuron’s position in the nerve cord. For instance, neurons of all types nearer to the worm’s head expressed some of the same genes, whereas neurons nearer to the tail expressed a distinct set of shared genes.
Among the genes that are expressed differently are the famous Hox gene family, known for their role in defining boundaries along the anterior-posterior axis early in development (they are the reason your arms aren’t legs!) Hox genes also encode powerful gene activators, so they likely regulate various genes important to neuron function, thus adding a key piece to the neuron identity puzzle.
As I complete my PhD, I am continuing to research this new dimension of motor neuron diversity, with the goal of untangling the intricate gene network that connects the ubiquitous Hox genes to specific motor neuron traits. Hopefully, this information can be useful in understanding how neurodegeneration occurs, and in developing much needed treatments.
This article was originally published on the BSD News website.